Electrochemical Sciences
In the recent years extensive collaborative experimental and theoretical work focussed on the electrochemical oxygen reduction reaction (ORR), a fundamental electrochemical reaction relevant in both corrosion as well as energy conversion processes.
At metal surfaces the ORR is the cathodic partial reaction driving many corrosion processes. On the other hand, the electrocatalysis of the ORR in fuel cells and air batteries is intensely studied with the aim of improving the kinetics and reducing the overpotential that limits the energy efficiency. Several cooperative research projects in the GO-Department have focussed on shedding light on the complex multi-step reaction mechanism that strongly varies with the electrocatalytic surface and reaction conditions.
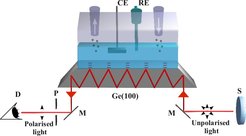
H2O2, a known side product or intermediate, offers the opportunity to selectively study the serial pathway, which was exploited in combined electrochemical and theoretical investigations [3-5]. The H2O2decomposition reactions on platinum were shown to be very fast (diffusion limited) for a wide range of potentials showing that the serial ORR pathway is possible even if H2O2 cannot be detected. Electronic structure calculations of the activation energies confirmed, that reaction pathways and kinetics of the peroxide decomposition are controlled by the oxidation state of Pt surfaces. Surface sensitive vibrational spectroscopy allows direct detection of ORR intermediates and thereby validation of the mechanism. A spectro-electrochemical ATR-IR setup has been developed and in a first application ORR on the model semiconductor surface n-Ge(001) has been studied.
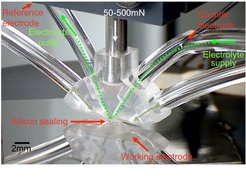
Within the last years, the newly developed and unique Scanning Flow Cell (SFC) coupled to the inductively coupled plasma mass spectrometer (ICP-MS) depicted in Fig. 2 has become a workhorse for high-throughput investigations of the stability of electrocatalysts, and the study of electrochemical processes such as corrosion or dealloying [6-8].
This has been utilized to increase the understanding of the (electro-) chemical dissolution of metals as a critical process for both, the design and synthesis of new catalysts and the prevention of their degradation during operation. Due to the wide parameter-space for material composition and structure as well as operation conditions fast screening techniques are necessary to obtain reliable information within a reasonable timeframe. For this purpose the scanning flow cell (SFC) automated high-throughput technique has been extended to enable the analysis of finely dispersed, porous catalyst materials. In cooperation with the industry partners the activity and stability of different catalysts for the application in polymer electrolyte membrane fuel cells has been a central aspect of the SFC work. Polymer electrolyte fuel cell technology is considered as one of the possible alternatives for electromobility applications, however the extensive use of and the degradation of Pt especially at the cathode still prevents its commercialization. Alloying with transition metals is a common strategy to generate highly active yet stable ORR electrocatalyst, as it lowers Pt loadings and also increases the activity due to ligand or strain effects. However, the instability arising from the electrochemical dissolution of the less noble metal, causing a decrease in the activity and poisoning of the membrane remains. On the other hand, dealloying before implementation in the membrane-electrode-assembly can be a useful tool in designing catalysts as it improves activity compared to conventional catalysts [9]. To follow the microstructural changes of Pt-based catalysts at a nanoscale level we employ the combination of conventional electrochemical techniques and the Identical Location TEM approach (IL-TEM). The latter provides insights in the dealloying pathways, size dependent morphological changes and degradation process. Extending the IL-TEM to more sophisticated characterization techniques (HAADF-STEM, EELS and tomography) allows an investigation also of the compositional variation on the sub-nanometre scale and the development of a 3D reconstruction of the particle dispersion. Combining this approach with conventional electrochemistry provides a complete description of the catalytic activity in relation with the surface structural characteristics.
The scientific aim is to understand the mechanisms that govern the corrosion of the materials in order to design catalysts with high stability without compromising activity. The SFC was complementarily used to characterize the early stage of dealloying of model Cu3Au and Cu3Pt single crystals for developing a fundamental understanding of dealloying processes. To keep in touch with real world applications, high-surface-area bimetallic nanoparticles like CuPt were studied in parallel. Selective leaching of less noble elements from such nanosystems results in formation of porous and/or core/shell structures preserving the increased activity towards such important reactions as electrochemical oxygen reduction.
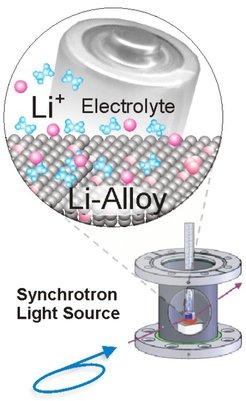
Currently, Li-ion batteries are the main alternative in use as energy storage in the automotive industry and in portable consumer electronics. The anode in Li-ion batteries represents a very reactive electrode, which often requires in-situ measurements to address the actual mechanisms at work during charging and discharging reactions. For the use with ionic liquids we have recently adapted and now intensively tested a UHV-compatible in-situ electrochemical X-ray diffraction chamber employed at synchrotron light sources. By their low interaction with matter especially high-energy photons in the range above 60 keV offer great possibilities for in-situ diffraction studies. Eventually replacing carbonaceous materials, silicon may become one of the most important anode materials in the future. Theoretically it can store many times more Li but structural and primarily volume changes pose currently still technical problems. Au nanoparticles are one way to obtain Si nanowires but the strong Au-Li signals obscure the interpretation of X-ray diffraction data from the Si nanowires. Here we started to examine pure Au model systems [10,11] with high-energy synchrotron light at the new light source PETRA3 in Hamburg and could unambiguously identify two metastable Au-Li phases, which constitute the main phases during cycling. Further work will focus on different Si-based materials and using the new Pulsed Laser Deposition system for producing thin film model electrodes.